Introduction
Biomass
Biomass conversion technologies
Mechanical conversion
Thermochemical conversion
Biochemical conversion
Conclusion and Future Outlook
Introduction
Energy consumption has steadily increased as a result of industrial advancement, driving the rising use of fossil fuels. This increase in fossil fuel usage has led to environmental pollution and increased CO2 emissions, contributing to global climate change. In a recent statement, the UN referred to the issue not as global warming but as “Global boiling” (Victoria Bisset, 2023). Nevertheless, global fossil fuel consumption continues to rise. Based on the primary energy supply, in 2019, oil accounted for 4572.7 million tons of equivalent (TOE), while coal and natural gas stood at 3765.1 million TOE and 3356.8 million TOE, respectively. In 2020, due to the global pandemic, these numbers decreased slightly to 4149.6 million TOE for oil, 3616.7 million TOE for coal, and 3287.0 million TOE for natural gas (Fig. 1). However, with the easing of the pandemic, fossil fuel consumption began to rise again. To address this climate crisis, the supply of renewable energy has also increased, growing from 616.8 million TOE in 2018 to 953.3 million TOE.
Renewable energy encompasses both new and regenerative energy sources. New energy refers to energy derived from the conversion of traditional fossil fuels or through chemical reactions such as hydrogen and oxygen to produce electricity or heat. This category includes hydrogen energy, fuel cells, and coal liquefaction and gasification. Renewable energy, on the other hand, involves converting and utilizing naturally replenishing sources such as sunlight, water, geothermal energy, precipitation, and organic materials. This category encompasses photovoltaics, solar thermal, wind, hydropower, marine, geothermal, bioenergy, and waste-to-energy sources.
The Republic of Korea heavily relies on thermal power generation for over 60% of its electricity production. Among the percentages, approximately 35% is generated through coal-fired power plants, but there is an ongoing shift towards using LNG for thermal power generation (Fig. 2 left). Although the use of renewable energy sources for electricity generation has been growing, with the generation capacity increasing from 27,874 GWh in 2017 to 40,041 GWh in 2021, this still constitutes only about 6.9% of the total power generation.
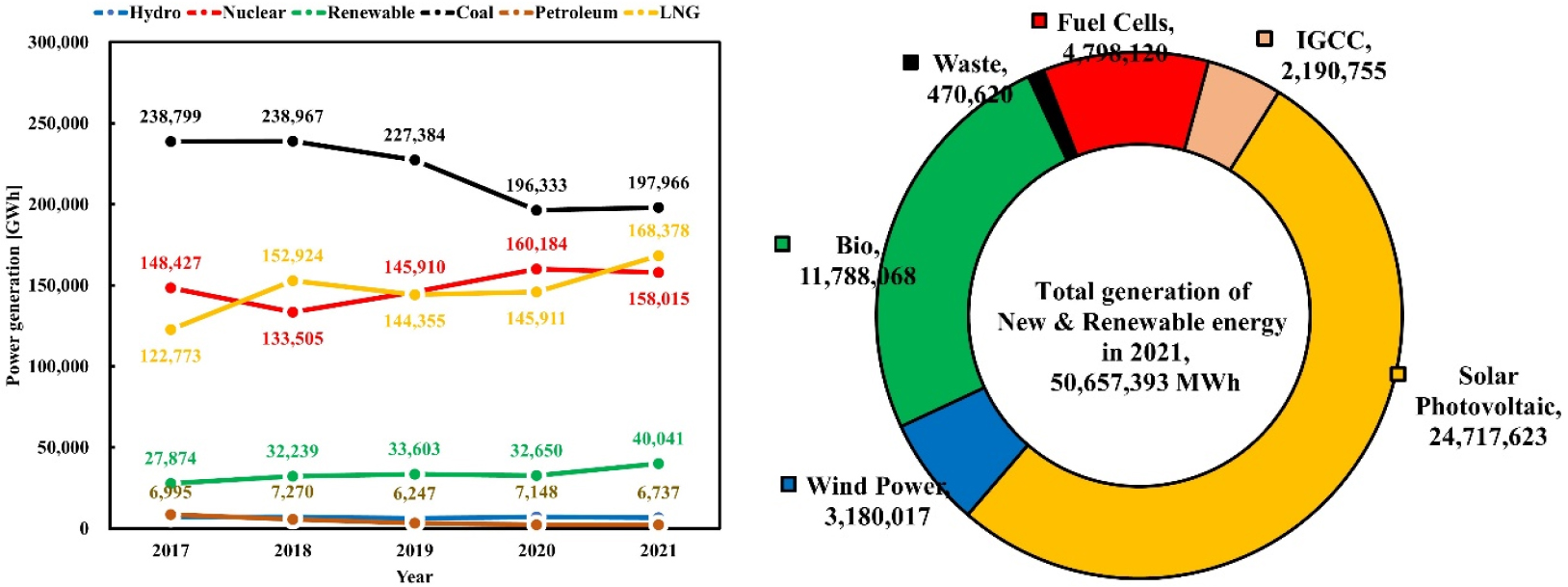
Fig. 2.
Power generation in Korea (left) and the generation of new and renewable energy (right) (Yang, 2022)
The “Act on Promotion of New Energy and Renewable Energy Development, Utilisation, and Distribution” was put in place to increase the variety of energy sources, ensure a steady supply of energy, encourage energy structures to be changed in ways that are better for the environment, and lower greenhouse gas emissions. Under the “Act on the Promotion of the Development, Use, and Diffusion of New and Renewable Energy”, power generation facilities with a capacity of 500 MW or more are obligated to supply a certain amount of electricity generated from renewable energy sources (Act On The Promotion Of Saving And Recycling Of Resources, 2017). As of January 2023, the Korean Ministry of Trade, Industry, and Energy announced proposed amendments to the “Act on the Promotion of the Development, Use, and Diffusion of New and Renewable Energy”. According to these proposed amendments, the mandatory supply ratio for 2023 will be reduced from 14.5% to 13.0%. However, it is expected to increase to 25.0% by the year 2030 (Act on the Promotion of the Development, Use, and Diffusion of New and Renewable Energy, 2023).
Due to the significant share of coal-fired power generation in the overall energy mix, there is a growing interest in utilizing bioenergy as a replacement. Specifically, there is high interest in woody pellets, which closely resemble traditional coal, as a feasible replacement for existing infrastructure. Among renewable energy sources, bioenergy accounts for approximately 26.99% (Fig. 2 right). Within bioenergy generation, 57.64% is produced using woody pellets, and this has been reported to be used for various industrial and commercial purposes (Yang, 2022). The global electricity production from renewable energy sources is projected to rise from 5,304,340 GWh in 2015 to 8,439,671 GWh in 2023. The bioenergy installed capacity is projected to rise from 90,101 MW in 2014 to 144,286 MW in 2022. However, the growth rate will be slower, with an estimated capacity of 148,840 MW in 2023. Power generation has been expected to have a gradual growth from 428,096 GWh in 2014 to 618,916 GWh in 2022 (International Renewable Energy Agency, 2024).
Biofuels derived from biomass are considered carbon-neutral due to their lower carbon footprint (Okolie et al., 2021). Biofuels emit CO2 during combustion; however, this CO2 is subsequently recycled through photosynthesis, making biofuels carbon-neutral. Biomass is crucial in the establishment of a circular economy, where biomass conversion is used to manufacture various value-added goods and chemicals through an integrated strategy (Sikarwar et al., 2021). The global biomass reserves have the capacity to generate 33,000 exajoules of energy, which is 80 times greater than the entire annual energy consumption globally (Tursi, 2019). It is important to highlight that biomass is presently not fully used for energy generation. Given the vast potential of biomass materials and their global accessibility, it is crucial to create efficient and environmentally-friendly methods for converting them into biofuels and valuable compounds (Cai et al., 2017).
Biomass
Biomass is a term that encompasses plant life synthesising organic matter using solar energy, along with animals, microorganisms, and other organic organisms that consume them as food. Biomass can be converted into solid, liquid, or gaseous biofuels. The advantages and disadvantages of biomass are summarised in Table 1 (Baxter, 2005; Dai et al., 2008; Darvell et al., 2010; Fatih Demirbas, 2009; Mckendry, 2002a; Vassilev et al., 2010, 2012, 2013a, 2013b, 2014, 2015).
Table 1.
Advantages and disadvantages of biomass
Biomass, with its advantages and disadvantages, is primarily classified into two main categories using two methods: by type and by generation.
First, the classification based on the type of biomass is summarised in Fig. 3. Biomass is broadly categorised into plant and animal sources. In the plant source, it is further divided into naturally grown biomass, residues, and energy crops. The residues category includes agricultural residues, forestry residues, industrial biowaste, and urban solid waste. Under agricultural residues, crop residues and unused forestry residues, among others, are included (Cardoen et al., 2015; Hays et al., 2005; Oh et al., 2019; Saleem, 2022). Industrial biowaste refers to organic waste generated across various industries, with a notable contribution from food factories and sewage sludge (Mishra et al., 2023; Zhuang et al., 2022). Municipal solid waste (MSW), which is commonly referred to as garbage, is a heterogeneous combination of materials originating from residential, commercial, and industrial sources (Blanco-Canqui, 2010; Sims et al., 2006; Zegada-Lizarazu and Monti, 2011). Energy crops refer to crops cultivated specifically for bioenergy production (Blanco-Canqui, 2010; Sims et al., 2006; Zegada-Lizarazu and Monti, 2011). However, it’s important to note that these crops are not intended for human consumption. They are typically characterised by their high biomass yield. In the case of animal derived biomass, it refers to biomass produced while raising livestock in places like barns. One of the primary sources for this category is animal manure (HE et al., 2016; Isemin et al., 2019; Manogaran et al., 2022). One of the other animal sources is carcasses (Tápparo et al., 2020; Xu et al., 2018; Zhang and Ji, 2015).
The second classification is categorization by generation (Fig. 4). Feedstock such as corn and sugarcane for energy generation consists mainly of starch (including sugar) and edible triglycerides in 1st generation (Hang and Woodams, 2001; Limtong et al., 2007). The straightforward process of hydrolysing glucose polysaccharides has made them a popular choice for producing sugar monomers. However, the utilization of edible food crops in biofuel and biochemical production has raised concerns about food prices and availability. This has led to the exploration of alternative non-edible feedstocks (Srinivasan, 2009). 2nd generation biomass is lignocellulosic biomass. Lignocellulosic biomass is considered a viable replacement for earlier generations of food crops, mainly due to its non-edible characteristics and its widespread availability at a low cost (Sathendra et al., 2022). Nevertheless, the significant obstacle to the utilisation of lignocellulosic biomass is the elevated crystallinity of cellulose, which contributes to recalcitrance and further complicates the process (Mosier et al., 2005). Also, the presence of lignin makes it difficult to access hemicellulose and cellulose, requiring various pretreatment methods to overcome this limitation (Tomás-Pejó et al., 2011). Algae represent the third generation of biomass. Researchers have classified algal feedstocks separately due to their high lipid yield and their ability to thrive on non-arable land. Algae can also adapt to various carbon sources, making them a versatile option (Wijffels et al., 2010; Zeng et al., 2011). Fourth-generation feedstock encompasses genetically modified organisms designed to improve biohydrogen production processes. Biomass derived from the second and third generations, following genetic enhancements, falls into the category of fourth-generation feedstock. But 3rd and 4th generation biomass are challenging techniques to use commercially (Goria et al., 2022). The economic viability of large-scale algae production facilities is still limited, particularly in the case of 4th generation biomass, due to factors such as limitations on genetic manipulation (Kumar and Verma, 2021; Mat Aron et al., 2020; Shokravi et al., 2021; Singh et al., 2021).
Biomass conversion technologies
Natural gas, or petroleum, has the advantage of being easy to transport and store and having a high calorific value. However, in order to use biomass as an alternative to the above fuels, the problems of low apparent density, energy density, and heterogeneity must be solved. Various conversion technologies for using biomass are being proposed. There are largely three categories of conversion methods: physical conversion using pellets, briquettes, etc.; biochemical conversion using enzymes or bacteria; and thermochemical conversion using heat. Fig. 5 demonstrates a schematic of biomass conversion technologies.
Mechanical conversion
Physical or mechanical conversion of biomass involves modifying biomass through a series of preliminary operations, including size reduction, drying, and densification. This procedure results in biomass taking on forms that possess enhanced characteristics compared to raw biomass. These improved properties include higher mass density, greater energy density, and increased resistance to moisture compared to the original biomass (Orisaleye et al., 2023). Commonly utilised biomass conversion techniques in this category encompass chipping, briquetting, pelleting, extraction, and distillation.
Extraction is a mechanical process used to produce oil from the seeds of various biomass crops, such as cotton and groundnuts. This method results in the extraction of oil as well as a residual solid known as “cake,” which can be used as animal feed (Kumar et al., 2015). Achieving complete oil extraction requires breaking down the cell walls, and this can be achieved using various mechanical or non-mechanical techniques (Halim et al., 2013; Makareviciene and Sendzikiene, 2022; Mckendry, 2002b). Steps in the extraction parameters’ optimisation have an impact on the effectiveness of the oil recovery process. This optimisation depends on factors such as the oil content in the plant material, the physical-chemical properties of the oil, and other related considerations.
Crushing oilseeds to extract the oil, steam distillation of the oil mixture, and vaporisation of essential oils are all steps in the distillation process. These vaporised oils are then collected, condensed, or cooled to obtain biooil (Lei et al., 2003; Šulgan et al., 2020). However, one limitation of the distillation method is its substantial energy requirements (Al-Hotmani et al., 2020). Distillation involves a high energy demand for heating the liquid, converting it into vapour, and ultimately condensing the vapour back into liquid at the condenser.
One of the disadvantages of biomass is its low density. To solve the problem, chipping can be one solution. Wood chips are finely crafted fragments of wood that are derived from logs and residual wood materials generated during the production of solid wood products like timber and plywood (Enforcement Decree of the Act on the Sustainable Use of Timbers, 2018). These chips are typically aimed to have dimensions of approximately 4-6 mm in thickness, 3-200 mm in length, and width (Fuller, 2004).
Also, densification can be widely used as a solution to low density. Densification refers to the process of crushing and pulverising biomass to produce a specific product using a specific mold. These mainly include pellets, and briquettes (Dinesha et al., 2019; Fuller, 2004; Kayo et al., 2013). The quality of briquettes is primarily assessed based on their density. Challenges associated with the disposal of agricultural and industrial biomass wastes can, in part, be alleviated by utilising them as biomass fuel in the form of briquettes (Roman et al., 2023; Sette et al., 2018). When produced cost-effectively and made readily available to consumers, biomass briquettes can act as supplements to traditional fuels such as firewood, charcoal, and kerosene for household cooking and agro-industrial operations. This helps reduce the high demand for other types of fuels (Obi, 2015). Briquettes offer several advantages over conventional firewood, including higher heat output, ease of use, cleanliness, and compact size. The compact size reduces the need for storage space and makes transportation more affordable and less resource intensive.
Pellets are compact, small cylindrical pieces measuring between 3 and 20 mm in length and 5 to 10 mm in diameter (Enforcement Decree of the Act on the Sustainable Use of Timbers, 2018; Park et al., 2020). These pellets are manufactured from finely ground wood. They are commonly employed as fuel by being automatically fed into burners due to their small and consistent size. However, it’s worth noting that energy is expended in the drying and grinding processes necessary for creating wood pellets. Consequently, wood pellets exhibit a lower ratio of energy produced to energy invested compared to wood chips. Nevertheless, wood pellets are a practical choice for efficient burning in smaller stoves in residential homes (Kayo et al., 2013).
Thermochemical conversion
Biomass is converted by a thermochemical process into a better fuel or a syngas that is high in hydrogen and can be used to produce chemicals using Fischer-Tropsch (F-T) synthesis (Choudhury et al., 2015). It has several important steps, such as drying, pyrolysis, changing between heterogeneous and homogeneous chars, and reforming reactions with pyrolysis materials (Zheng et al., 2022). From a business point of view, this method appears advantageous because it provides more options, allows the selection of specific products, accelerates the conversion process, and could lead to new markets for byproducts (Sarker et al., 2021). However, thermochemical technology often requires substantial process equipment and operates under high-temperature and high-pressure conditions, which can be costly (Nizami et al., 2017). The main challenge in thermochemical processes is the significant investment required for infrastructure compared to the low cost of the feedstock. Therefore, to achieve cost-effectiveness, additional research and development efforts are necessary (Castaldi et al., 2017). Currently, the majority of biomass thermochemical conversion methods include torrefaction, pyrolysis, gasification, and combustion.
Torrefaction
Torrefaction is a thermal pretreatment method for biomass. In this method, untreated biomass is heated at temperatures between 200°C and 300°C in an environment low oxygen levels. The objective of this process is to improve the quality of solid biomass fuel. The simplified chemical process equation for the pyrolysis process is as Eq. (1).
As torrefaction technologies have been developed, various types of torrefaction processes have been introduced, as summarised in Fig. 6.
Nitrogen is often used as the carrier gas in lab experiments to create an atmosphere that doesn’t react with oxygen during the torrefaction process. In non-oxidative torrefaction, both nitrogen (Chen and Kuo, 2010; Nepal et al., 2022) and carbon dioxide (Hernández et al., 2017; Saadon et al., 2014) have been utilised as carrier gases, with nitrogen being the more commonly used for sweeping biomass materials during thermal pretreatment. Also, torrefaction was carried out by sealing to prevent the inflow of oxygen (Kim et al., 2022; Oh et al., 2019; Park et al., 2021). On the other hand, for oxidative torrefaction, attempts have been made using air (Chen et al., 2014; Wang et al., 2013, 2021; Zhang et al., 2019a), flue gas (Chen et al., 2014; Wang et al., 2013, 2021; Zhang et al., 2019a), and other gases containing varying oxygen concentrations (Rousset et al., 2012a, 2012b; Wang et al., 2018; Zhang et al., 2019a) as carrier gases for biomass pretreatment. Due to the presence of oxygen and the occurrence of exothermic reactions during thermal degradation, oxidative torrefaction exhibits a higher reaction rate compared to non-oxidative torrefaction (Park et al., 2023a, 2023b), resulting in shorter torrefaction durations. Using air or flue gas for biomass torrefaction can lead to reduced operating costs since there’s no need for nitrogen separation from the air. However, oxidative torrefaction yields lower solid content compared to non-oxidative torrefaction (Brachi et al., 2019; Chen et al., 2020; Park et al., 2023a, 2023b; Zhang et al., 2019b). It has also been observed that the HHV of torrefied biomass decreases as the oxygen concentration increases when torrefied at a temperature of 300°C (Wang et al., 2018). A comparison between oxidative and non-oxidative torrefaction is summarised in Table 2.
Table 2.
Comparison between non-oxidative and oxidative torrefaction
Biomass can be processed in moist environments, such as water and diluted acid, to enhance biomass properties, resulting in the production of a substance known as “hydrochar.” In the case of biomass subjected to torrefaction in hot compressed water or hydrothermal conditions, it has been observed that there is minimal biomass reaction occurring in liquid water at temperatures below 180°C (Lynam et al., 2011). As a result, wet torrefaction is typically conducted within a temperature range of 160°C to 260°C, with reaction times varying from 5 to 240 minutes (Chen et al., 2012; Lynam et al., 2011) and pressures of up to 5 MPa. As the temperature of the water increases, its properties undergo significant changes, including alterations in the dielectric constant, ion products, density, viscosity, and diffusivity (Bach and Skreiberg, 2016). These transformations facilitate biomass degradation within the liquid or aqueous phase. Consequently, wet torrefaction is commonly carried out under conditions resembling the subcritical state. Additionally, the incorporation of substances like sulfuric acid (Gan et al., 2020a, 2020b) and acetic acid (Lynam et al., 2011) is another practice in this process. Table 3 summarises the differences between dry and wet torrefaction.
Table 3.
Comparison between dry and wet torrefaction
Surface torrefaction, which addressed the shortcomings of the above two processes, is also presented (Kim et al., 2021). It can solve the high mass loss, which is a disadvantage of the existing two processes, and has the advantage of a relatively shorter process time. However, disadvantages such as a relatively low increase in heat generation are also pointed out.
Pyrolysis
Pyrolysis is the process of breaking down biomass through heat in the absence of oxygen. This occurs within a temperature range of 300-800°C (Angin, 2013; Demirbaş and Arin, 2002; Paul and Serpil, 1996; Tomczyk et al., 2020). During pyrolysis, biomass is transformed into biooil, biochar, and syngas. If the pyrolysis process is employed to generate charcoal or biochar rather than biooil, the proportions of these products depend on factors like the type of biomass, the reactor used, the operating temperature, and the heating rate (Xiong et al., 2018). Biochar has various applications, such as serving as a nutrient carrier in agriculture and an effective carbon storage medium (Park et al., 2023c). The following equation provides a concise overview of the reactions occurring in various thermal conversion processes of biomass.
As shown in this Eq. (2), the reactant is biomass, which is made up of hemicellulose, cellulose, lignin, and smaller amounts of other organic substances.
Pyrolysis can be categorised into three methods based on the heating rate: slow, intermediate, and flash pyrolysis. The descriptions for each pyrolysis process have been summarised in Table 4. Slow pyrolysis involves heating biomass to 300 - 700 °C at a rate less than 50 °C/min. This process takes several hours to convert biomass into bioproducts (Jonsson, 2016). When the focus is primarily on char, it is commonly referred to as carbonisation (Boateng, 2020). Intermediate pyrolysis falls between fast pyrolysis and slow pyrolysis on the pyrolysis spectrum. It exhibits a favourable product distribution, making it suitable for the simultaneous production of biochar, biooil, and gas (Zaini et al., 2021a). In contrast to other methods, intermediate pyrolysis is known for its versatility in handling diverse materials, offering excellent product distribution, the ability to separate biooil into two distinct phases, ensuring high product quality, and yielding dry biochar (Hornung, 2013; Hornung and Schröder, 2014a, 2014b; Ju et al., 2018; Morgano et al., 2018; Tan et al., 2021; Yang et al., 2017; Zaini et al., 2021b). Flash pyrolysis is widely used due to its shorter conversion time. It operates with a heating time of less than 2 seconds and a heating rate of more than 60,000 °C/min (Bridgwater and Peacocke, n.d.; Pattiya, 2017). The utilisation of heated fluidization sand enable performance at a comparatively moderate temperature range of 400-600°C (Chen et al., 2022; Choi et al., 2020). The liquid pyrolysis oil produced is most often termed biooil. The resulting product distribution from fast pyrolysis typically falls within the range of 60-75% biooil, 15-25% biochar, and 10-20% syngas (Balat et al., 2009; Boateng, 2020; Bridgwater and Peacocke, 2000; Guda et al., 2015; Jonsson, 2016; Kim et al., 2012; Park et al., 2011; Pattiya, 2017). However, fast pyrolysis requires biomass to be pre-shredded for uniform heating.
Table 4.
Pyrolysis conditions
Gasification
Gasification is a thermochemical process where fuel reacts with a gasification agent, resulting in the production of syngas, which is alternatively known as producer gas, product gas, or simply syngas (Basu, 2018). This syngas primarily consists of CO, H2, N2, CO2, along with some hydrocarbons like CH4, C2H4, C2H6, and so on. In addition, trace amounts of H2S, NH3, and tars may also be present (Balu et al., 2015; Dai et al., 2015; De Oliveira et al., 2018; Gao et al., 2016; Ghenai and Inayat, 2019; Lozano and Lozano, 2018; Ruiz et al., 2013; Shah et al., 2023; Yao et al., 2018; Zhang et al., 2015). In a broader context, biomass gasification involves the thermochemical transformation of organic feedstock within a high-temperature environment (Choi et al., 2023; Ghenai and Inayat, 2019; Maneerung et al., 2018). This process allows biomass to be converted not only into syngas for energy production but also into various chemicals, such as methane, ethylene, adhesives, fatty acids, surfactants, detergents, and plasticizers (Lozano and Lozano, 2018). The reactions (Eqs. (3), (4), (5), (6), (7), (8)) that commonly take place during the gasification of solid carbon include (Acevedo et al., 2018; Choi et al., 2023):
Combustion
Combustion, arguably one of the earliest methods for harnessing biomass, traces its roots back to the dawn of civilization, when humans discovered fire (Euh et al., 2017). From a chemical perspective, combustion represents an exothermic reaction involving the interplay of oxygen and hydrocarbons within biomass. In this process, biomass undergoes oxidation, resulting in the formation of two major stable compounds: H2O and CO2 (Jenkins et al., 2019). Currently, the heat released through this reaction stands as the primary source of human energy consumption, accounting for over 90% of energy derived from biomass. Heat and electricity stand out as the two primary forms of energy obtained from biomass. Biomass continues to serve as a valuable source of heat for cooking and heating, especially in rural regions. Additionally, steam produced by the combustion of biomass in boilers fuels district and industrial heating systems (Demirbas, 2004). In several countries with frigid climates, the provision of warmth is facilitated through the utilisation of pellet stoves and log-fired fireplaces. Furthermore, the combustion of biomass has the capacity to generate electrical energy, serving as a fundamental pillar of modern economic endeavours. The most common approach involves generating steam by burning biomass in a boiler and then utilising a steam turbine to produce electricity (Fernandes et al., 2016; Nunes et al., 2016; Ryu et al., 2006). Biomass is employed either as a standalone fuel source or in conjunction with fossil fuels within a boiler. The latter option is gaining popularity as it represents a swift and cost-effective means of reducing carbon dioxide emissions from existing fossil fuel plants (Basu et al., 2011). Biomass combustion can generate hot gases at temperatures of up to 1000°C. However, efficient combustion of biomass is achievable only when its moisture content is below 50% (Tripathi et al., 2016).
Biochemical conversion
Various products are made from biomass by microorganisms breaking it down. These products include hydrogen, biogas, ethanol, acetone, butanol, organic acids such as pyruvate, lactate, oxalic acid, levulinic acid, and citric acid, as well as 2,3-butanediol, 1,4-butanediol, isobutanol, xylitol, mannitol, and xanthan gum (Becker et al., n.d.; Chen and Qiu, 2010; Cheng et al., 2021; Faria et al., 2009; Karaffa et al., 2001; Ndaba et al., 2015; Syu, 2001; Zhao et al., 2020). There are three main technologies employed for the biochemical conversion of biomass into biofuels and bioproducts: anaerobic/aerobic digestion, fermentation, and hydrolysis (Bharathiraja et al., 2018; De Vrieze and Verstraete, 2016; Koyama et al., 2014; Li et al., 2017; Pasalari et al., 2021; Ross et al., 2002).
Digestion
Anaerobic digestion (AD) involves a series of interconnected biological reactions in which organic matter is transformed into methane (CH4), carbon dioxide (CO2), and anaerobic biomass in the absence of oxygen (Yilmaz and Selim, 2013). Usually, the four stages (hydrolysis, acidogenesis, acetogenesis, and methanogenesis) of anaerobic digestion are required, ultimately resulting in the generation of carbon dioxide and methane as organic matter breaks down (Kim and Kim, 2019; Li et al., 2015; Lohani and Havukainen, 2018; Wang et al., 2023). The CH4 yield per unit area is primarily used to regulate the energy efficiency of a particular feedstock. This yield varies significantly between different organisms and is influenced by factors such as growth, geographic location, and inputs like water and manure, even among species that are otherwise similar.
On the other hand, in aerobic composting or digestion, microorganisms utilise oxygen from the air to break down biomass into heat, CO2, and a solid product. Under aerobic conditions, organic material undergoes oxidation, leading to the production of substances such as nitrate, phosphate, and carbon dioxide (Martín et al., 2015). This process transforms organic waste biomass into stable humus through the action of microorganisms in specific environmental conditions. It is commonly employed in the treatment of biomass waste (Jia et al., 2021; D. Wu et al., 2022). Compounds containing carbon and nitrogen are readily converted and serve as energy and protein sources for the microbes during the composting process. Microbial activity in aerobic digesters is usually vigorous, resulting in shorter retention times when producing biogas. Aerobic digestion is commonly used as a preliminary step to separate or eliminate the organic components from oil effluents (Ohimain and Izah, 2017). These treatments can be carried out at room temperature (25°C-35°C), but it’s essential to adjust the pH for their effectiveness (Gunay and Karadag, 2015).
Hydrolysis
The process of hydrolysis breaks down the β-1,4-glycosidic bonds in cellulose into simpler sugars, which are then used to make ethanol (Sukumaran et al., n.d.). Various biomass hydrolysis techniques, including both acid and enzymatic hydrolysis, are employed to transform the raw materials into straightforward sugars (Wu et al., 2008; Zhou et al., 2011). The mechanism of hydrolysis is depicted in Fig. 7.
Enzymatic hydrolysis utilises enzymes to dismantle the complex chemical composition of lignocellulose carbohydrates (Gnanasekaran et al., 2023; Huang et al., 2022; Saini et al., 2022; Walker and Wilson, 1991; Yang et al., 2011). These enzymes represent an eco-friendly approach to reducing waste production and energy consumption, making them essential for the establishment of cost-effective and sustainable biorefineries (Liguori and Faraco, 2016). The effectiveness of enzymatic hydrolysis primarily hinges on the accessibility and potency of enzymes, which are closely tied to the chemical characteristics of biomass (Zhu et al., 2008).
Acid-based hydrolytic methods have been used on various materials, such as softwood and hard woods, as well as agricultural waste. These methods use acid catalysts like sulfuric acid (H2SO4), hydrochloric acid (HCl), and nitric acid (HNO3). (Huang et al., 2022; Kumar et al., 2019; Park et al., 2015; Saini et al., 2022; Walker and Wilson, 1991; Yang et al., 2011). One significant drawback of the acid hydrolysis pretreatment method is its association with corrosion problems, necessitating the use of costly materials for equipment construction and operation. Furthermore, employing acid for biomass pretreatment has historically been linked to the generation of degradation byproducts like furfural, 5-hydroxymethylfurfural (HMF), and acetic acid, which can hinder subsequent fermentation processes (Iranmahboob et al., 2002; Jennings and Schell, 2011; Kang et al., 2018; Lenihan et al., 2010; Mosier et al., 2005; Rajan and Carrier, 2014; Świątek et al., 2020; Wang and Copeland, 2015; Zheng et al., 2009).
Fermentation
The fermentation process involves the conversion of biomass into valuable bioproducts like bioethanol, biohydrogen, and biogas using microorganisms such as bacteria, fungi, and yeast (Hossain, 2019). Especially in the case of fermentation, it is primarily applied to starch- or sugar-based crops such as corn and sugarcane. Ethanol fermentation is known as one of the oldest and most important fermentation processes (Li et al., 2023). Three common methods are used for bioethanol fermentation: simultaneous saccharification and fermentation (SSF), separate hydrolysis and fermentation (SHF) and simultaneous saccharification and co-fermentation (SSCF) (Das et al., 2023a). The process of each fermentation was summarised in Fig. 8. The main advantage of the SSF process over separate hydrolysis and fermentation is that it allows for high substrate concentrations, long residence times, and high enzyme concentrations to be used in the same reactor. However, the main disadvantage is the product inhibition caused by the accumulation of glucose during the hydrolysis step (Kanagasabai et al., 2019; Wood et al., 1997). In SHF, the hydrolysis of lignocellulosic materials and the fermentation of ethanol occur as distinct processes. However, a significant drawback of SHF is that the sugars released, especially cellobiose and glucose, have an inhibitory effect on cellulase activity (Taherzadeh and Karimi, 2007). SSCF refers to the simultaneous fermentation of both glucose and, primarily, xylose. This is advantageous in terms of minimising the final enzymatic hydrolysis. This makes it possible to convert lignocellulosic biomass, especially xylose-rich biomass such as xylose-rich hardwood and agricultural residues (Bondesson and Galbe, 2016; Broda et al., 2022; Das et al., 2023b; Dong, 2011; Koppram et al., 2013; Moreno et al., 2013). However, one challenge with SSCF is the need for microorganisms capable of fermenting not only glucose but also xylose. Also, the ethanol yield is lower compared to SSF (Broda et al., 2022; Liu et al., 2019; Liu and Chen, 2016; Olofsson et al., 2010).
Conclusion and Future Outlook
This study highlighted the three conversion processes’ potential—physical, thermochemical, and biological—as well as the diversity of biomass. We can draw the conclusion that these biomass technologies offer both significant advantages and drawbacks. Advantages of using different kinds of biomass and conversion techniques include lower pollution levels in the environment and the creation of costly renewable energy sources. Physical conversion, on the other hand, requires the usage of a variety of biomass because the amount of biomass that can be utilised is limited. Specifically, instead of pelletizing wood, there is an urgent need to employ solid biomass such as MSW and agricultural by-products. Even if a lot of technologies are being developed, thermochemical conversion still has drawbacks such as complexity, time commitment, low efficiency, and lack of economic viability. Recent developments in machine learning must be used to improve this. By doing this, ideal conditions for processing biomass will be found and implemented in the field, leading to the development of a biomass conversion technology that is both resource- and environmentally-efficient. Furthermore, there is an increasing amount of study being done on the production process of hydrogen, which is a new energy source that might replace existing by-product hydrogen with green hydrogen.
The biochemical conversion process will necessitate the advancements in technology in order to address the most pressing ethical issues related to food and energy sources. Nowadays, maize and sugarcane are the main crops used to make bioethanol. Optimising the process of turning inedible lignocellulose into biomass or into bioethanol, propanol, and butanol utilising microalgae is necessary to improve this.
Research is vital, but so is disseminating information about the advantages of producing renewable energy from biomass, which satisfies several of the 2030 Sustainable Development Goals (SDGs) that were established by the UN General Assembly in 2015.